Detection and limit test of diethylene glycol and ethylene glycol impurities in syrup by HPTLC
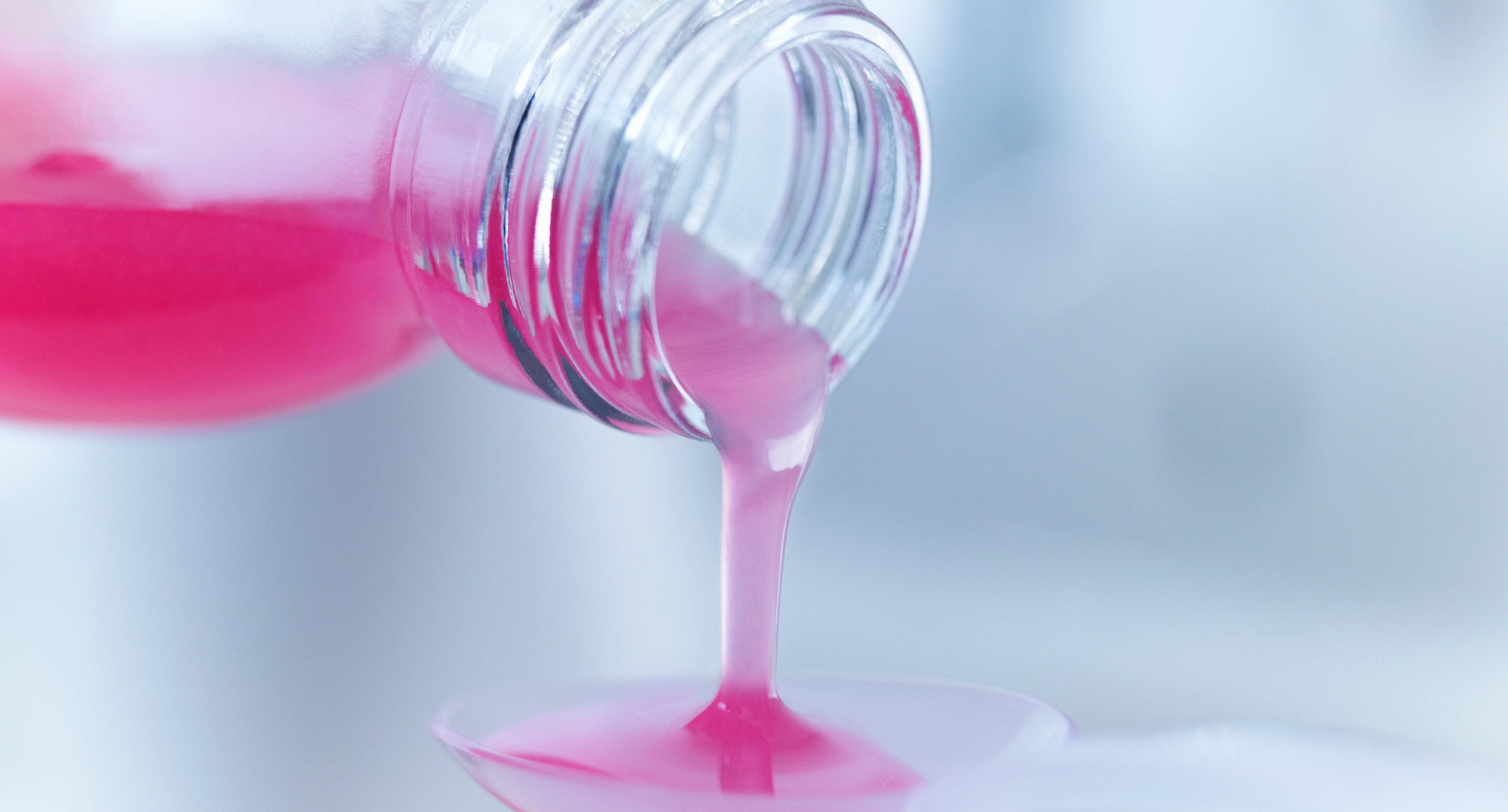
Detection and limit test of DEG and EG impurities in syrup products
Introduction
In 2023, the Food and Drug Administration (FDA) issued guidance to alert pharmaceutical manufacturers, compounders, repackers, and suppliers about the potential public health risks associated with diethylene glycol (DEG) and ethylene glycol (EG) contamination. This concern was underscored by multiple incidents reported in 2022 and 2023, where contaminated oral liquid drug products were identified in various countries. For instance, Indonesian health authorities detected DEG and EG in a propylene glycol excipient used in the production of oral liquid medications [1]. To mitigate such risks, the United States Pharmacopeia (USP) has established a safety limit for DEG and EG, requiring that their levels in excipients used for pharmaceutical formulations do not exceed 0.10 % as part of identity testing.
This guidance aims to facilitate the detection of DEG and EG-contaminated drug components, helping to prevent further poisoning incidents. The HPTLC method, adapted from a TLC approach [2], has been optimized to enhance sensitivity and reproducibility.
Sample preparation
1.0 g of syrup sample is dissolved in 10.0 mL of methanol, vortexed for 60 seconds, sonicated for 10 min, and centrifuged for 5 min at 5000 rpm. The supernatant is used as test solution.
Standard preparation
Reference solutions: 0.025, 0.0375, 0.05, 0.075 and 0.100 mg/mL of a 50:50 (m/m) DEG/EG mixture in methanol.
Chromatogram layer
HPTLC silica gel 60 F254 plates (Supelco) are used.
Sample application
Syrup samples and reference solutions are applied as 8.0 mm bands with the Automatic TLC Sampler (ATS 4), 15 tracks, band length 8.0 mm, distance from the left edge 20.0 mm, track distance 11.4 mm, distance from the lower edge 8.0 mm.
Chromatography
Plates are developed in the ADC 2 with chamber saturation (with filter paper) for 20 min and after activation at 33 % relative humidity for 10 min using a saturated solution of magnesium chloride, development with acetone – 25 % ammonia solution – toluene – water 85:1:5:9.5 (V/V) to a migration distance of 70 mm.
Post-chromatographic derivatization
After development, chemical derivatization is performed using potassium permanganate (KMnO4) reagent (dissolve 125 mg of sodium hydroxide, 9.9 g of sodium carbonate decahydrate, and 1.5 g of potassium permanganate in 200 mL of water). The plates are sprayed with 3.0 mL of derivatization reagent using the CAMAG Derivatizer (yellow nozzle, spraying level 3) and then dried in cold airflow for 4 min.
Documentation
Images of the plate are captured with the TLC Visualizer 2 in UV 254 nm (Detection A) prior to derivatization, and in white light reflection WR (Detection B) and white light reflection and transmission WRT (Detection C) after derivatization. Documentation is performed 30 minutes after the derivatization process.
Densitometry
Fluorescence measurement is performed with the TLC Scanner 4 and visionCATS at 520 nm (deuterium lamp), slit dimension 5.0 x 0.2 mm, scanning speed 20 mm/s (Detection D and without filter). Peak profiles from scanning densitometry (PPSD) were analyzed with visionCATS software 4.1.
Results and discussion
The results confirm the effective application of HPTLC in detecting excipients such as sorbitol, glycerol, diethylene glycol, ethylene glycol, and polypropylene glycol. The method yielded clear and reproducible chromatographic fingerprints, with the DEG/EG mixture exhibiting a unique RF value.
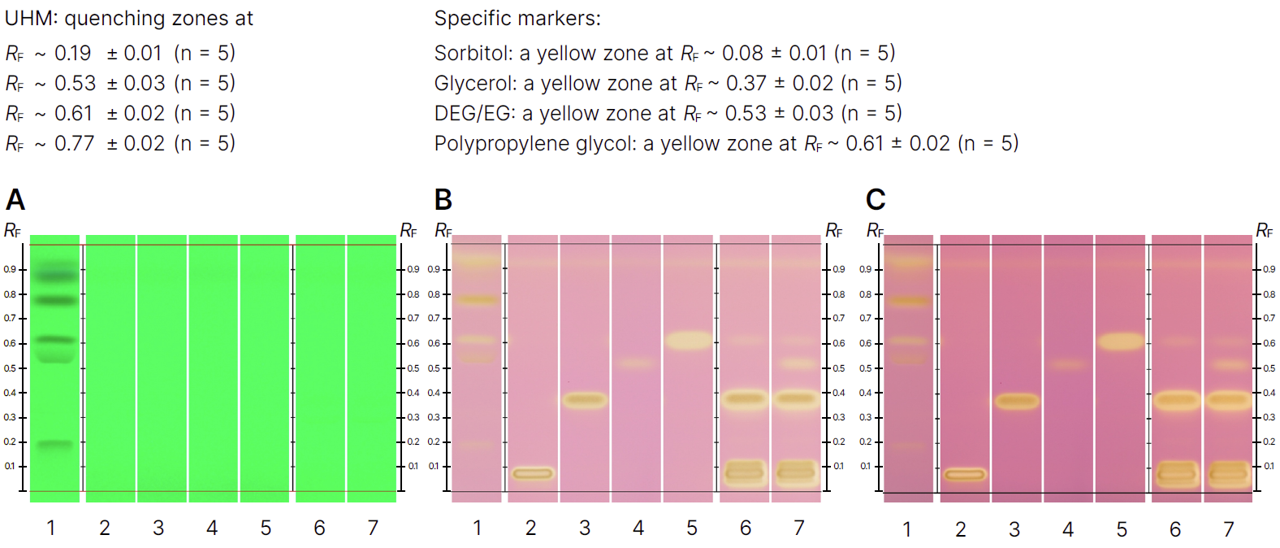
HPTLC fingerprints in UV 254 nm (A) prior to derivatization, white light in reflection (B) and white light in reflection and transmission (C) after derivatization. Track 1: UHM; track 2: sorbitol (1.0 mg/mL); track 3: glycerol (0.5 mg/mL); track 4: DEG/EG (0.1 mg/mL); track 5: polypropylene glycol (0.5 mg/mL); track 6: syrup 1 (100 mg/mL); track 7: syrup 2 spiked with 0.1 % DEG/EG (100 mg/mL).
When using profiles from scanning densitometry (PPSD) at 520 nm (detection D), the calibration curve was computed using a linear-2 function over a range of 50 ng to 200 ng. The limit of detection (LOD) for DEG/EG is 21 ng and the limit ofquantification (LOQ) is 45 ng.
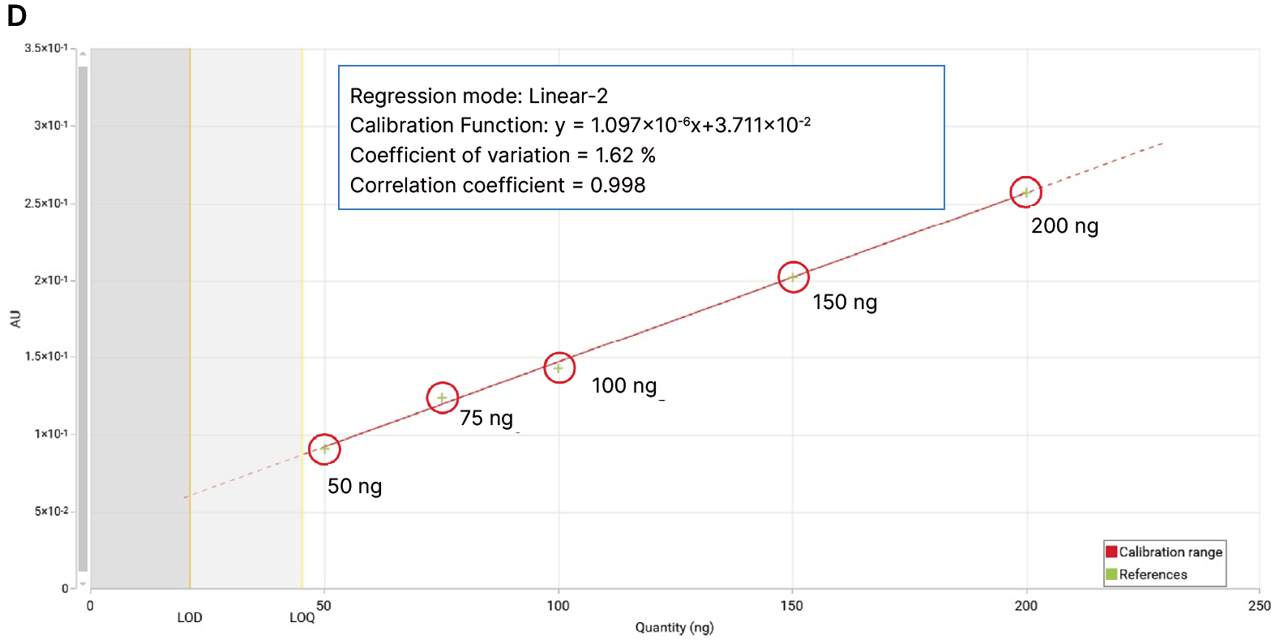
5-point calibration curve of DEG/EG (D) (from left to right, 50 ng, 75 ng, 100 ng, 150 ng, 200 ng, red circles) generated from peak profiles from scanning densitometry (PPSD) at 520 nm.
Two syrup samples were spiked with a mixture of DEG/EG. Using the reference solution prepared at 0.1 mg/mL as the limit test, a single-point calibration curve (linear-1) can be applied.
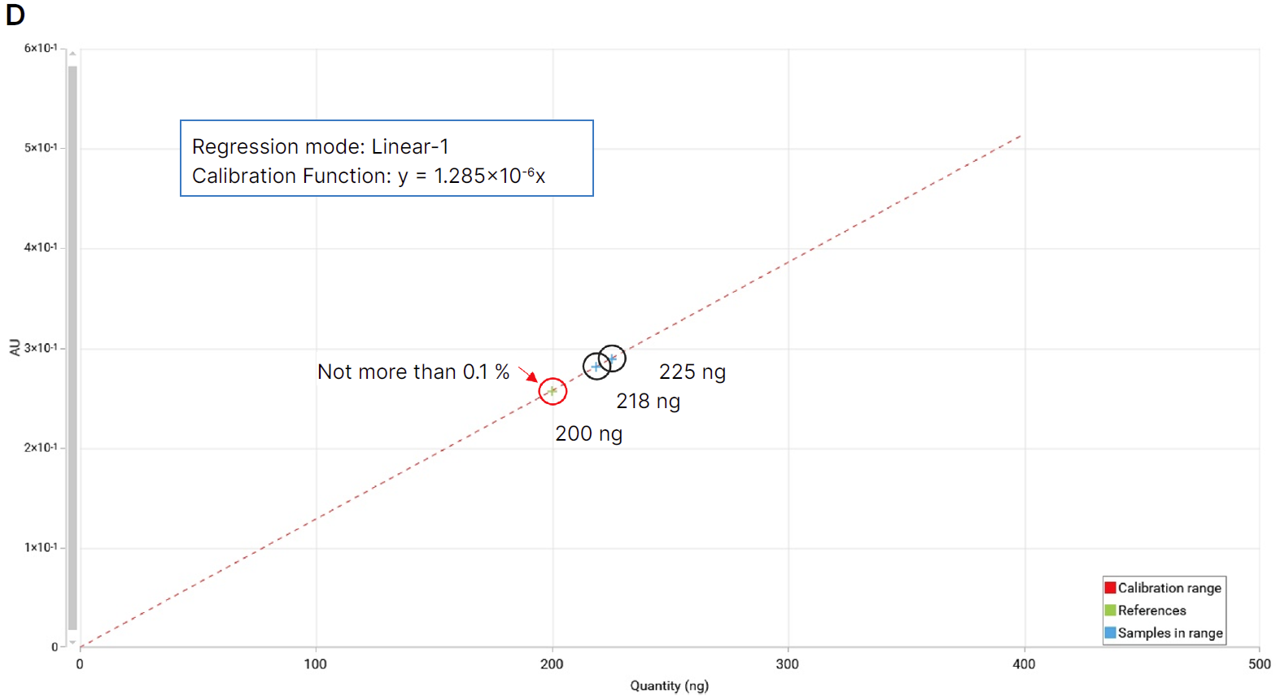
Single-point calibration (D), reference solution (0.1 mg/mL, red circle), samples above limit (100 mg/mL, black circles).
Conclusion
By optimizing the method for HPTLC, the limit test set at 0.1 % of DEG/EG in raw material and oral liquid formulations is easily achieved and can even be lowered to 0.03 %.
Literature
[1] Food and Drug Administration, Testing of Glycerin for Diethylene Glycol, (2023)
[2] A Concise Quality Control Guide on Essential Drugs and other Medicines, Special Edition, Diethylene glycol and ethylene glycol as impurities in liquids for oral use, (2024)
[3] T. K. T. Do et al., J Chrom. A (2021) 1638
Contact: Sonja Drobnjak, CAMAG, Sonnenmattstrasse 11, 4132 Muttenz, Switzerland, sonja.drobnjak@camag.com